Regular Articles
Vol. 16, No. 8, pp. 53–61, Aug. 2018. https://doi.org/10.53829/ntr201808ra1
Cell Encapsulation and 3D Self-assembly Using Multi-layered Polymeric Thin Films
Tetsuhiko Teshima, Hiroshi Nakashima, Yuko Ueno,
Satoshi Sasaki, Calum S. Henderson, and Shingo Tsukada
Abstract
Multi-layered thin films are spontaneously folded to form three-dimensional (3D) geometries. In this study, we demonstrate that polymeric thin films are self-folded to encapsulate cells. The films consist of two types of polymers with different mechanical stiffnesses; thereby, the rolled-up 3D tubular architectures with controllable diameters are fabricated based on the strain engineering. A batch release of sacrificial layers forms the multiple cells wrapped in rolled-up films, leading to artificial reconstruction of fiber-shaped cellular 3D constructs with the intrinsic morphologies and functions of living tissues. This system can potentially provide 3D biointerfaces that are necessary for the reconstruction and assembly of functional tissues and implantable tissue grafts.
Keywords: self-folding, polymeric thin film, tissue regeneration
1. Introduction
Three-dimensional (3D) biocompatible structures are strongly required for providing interfaces with cells to achieve cell-based assay [1], tissue engineering [2], and transplantation therapeutics [3]. Above all, the molded structures composed of non-cytotoxic polymer can produce ex vivo 3D cellular constructs with intrinsic cell-cell interactions, morphologies, and functions due to high elasticity and biocompatibility [4]. Although a variety of top-down fabrication processes such as laser micromachining or 3D printing techniques have been conventionally proposed, it is technically difficult to construct minute polymeric structures with confined 3D geometries.
To achieve 3D bottom-up fabrication, bimetal film has been utilized for self-assembly of 3D structures with precise geometry [5], which achieves curved bilayer films with heterogeneous mechanical properties. This system enables the assembly of a variety of 3D structures including pyramidal [6], tubular [1], and helical structures [7].
However, this bilayer mechanism is not sufficiently applied to polymeric 3D geometries due to the difficulty of creating polymeric bilayers. A 3D bottom-up fabrication of polymer 3D structures would provide an interface with cells to build 3D cell-laden architectures. Thus, an alternative method to produce a polymeric bilayer with heterogeneous mechanical properties is highly necessary. Cell-friendly polymers are surely applicable for ex vivo 3D cellular architectures with cell-cell connections, intrinsic morphologies, and various physiological functions [8].
In this study, we found that multi-layered polymeric films with heterogeneous mechanical properties can form self-folded rolled shapes (micro-rolls) [9]. Integrating the films with the non-cytotoxic batch release of a hydrogel-based sacrificial layer [10] makes it possible to autonomously fold the films into cylindrical shapes based on differential strain gradients that depend on the film thickness. Various 3D cell-laden microstructures were formed from two-dimensional (2D) geometrical micro-patterns, enabling the embedded cells to migrate and connect with each other. Within a micro-cavity, the cells tend to form the desired 3D architectures and synchronize their behavior. We also used various cell-lines and primary cultured cells to demonstrate that they can reconstitute the intrinsic cellular morphologies and functions. This system is potentially applicable to realizing 3D biointerfaces for such applications as the reconstruction of functional tissues and implantable tissue grafts.
2. Materials and methods
We fabricated multi-layered films composed of mechanically stable and biocompatible polymers, namely, poly(chloro-p-xylylene) (parylene-C) [10, 11] and silk fibroin [12] on an underlying sacrificial layer. We selected alginate hydrogel (calcium alginate (Ca-alginate)) as the sacrificial layer since it is dissolved with chelate agents. Thus, it achieves a spontaneous and non-cytotoxic batch release process with arrayed cell-laden films. As the film components, we used silk fibroin extracted from Bombyx mori [13] and parylene-C thanks to their mechanically robust features, high optical transparency, and excellent biocompatibility.
The fabrication process is shown in Fig. 1 [9]. First, sodium alginate was coated on the glass substrates and then immersed in calcium chloride to hydrogelate alginate with calcium ions (Ca2+) (Step 1). Next, the aqueous silk fibroin was coated and immersed in methanol for gelation in accordance with previously reported protocols [12] and laminated with a parylene layer by chemical vapor deposition (Step 2). Then photolithographical micro-patterning of the photoresist was carried out (Step 3). Thereafter, the trilaminar films consisting of alginate hydrogel, silk fibroin, and parylene were etched with oxygen plasma through a photoresist mask to fabricate a micro-patterned film array (Step 4). When the Ca-alginate layer was dissolved by adding ethylenediaminetetraacetic acid (EDTA), it gradually shrank from the edges and released the remaining bilayers as shown in Fig. 1 (Steps 5–8). SEM (scanning electron microscopy) images show identifiable trilaminar films with highly defined geometries (Fig. 2).
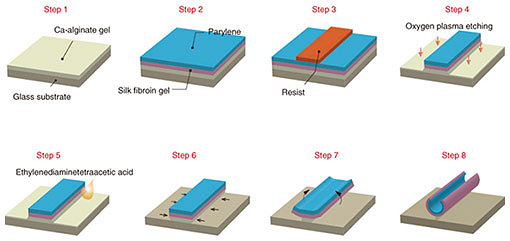
Fig. 1. Self-assembling thin film fabrication process. (Steps 1–4) Three layers of silk fibroin gel, parylene, and Ca-alginate gel are laminated and then processed using lithography to form an arbitrary 2D micro-pattern. (Steps 5–8) The Ca-alginate gel layer is removed with ethylenediaminetetraacetic acid to release the thin film from the
substrate.
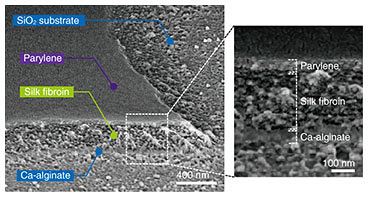
Fig. 2. Low-magnification SEM images of micro-patterned films on glass substrate. The white box indicates enlarged areas. Reprinted from Teshima et al. [9]. Copyright 2017.
3. Results
The self-folding process of the films and the manipulation and reconstruction of cells are explained in this section.
3.1 Self-folding of polymeric bilayer film
The combination of silk fibroin with conformally deposited parylene-C functions as a mechanically heterogeneous bilayer [9]. After the Ca-alginate layer was dissolved by EDTA, the released bilayer films transformed into controlled 3D geometries (Fig. 3(a)). This dissolution initiates the formation of tubular structures through self-folding. The curvature radius of the self-folded micro-roll reaches an equilibrium value, which is delayed by more than 10 s after the dissolution of the sacrificial layer. Confocal reconstructed images show that the dimensions of the micro-patterns had a noticeable effect on the diameter of the resulting tubular structures.
In addition to the cylindrical shape, various 3D geometries can be controlled and self-folded depending on the 2D micro-pattern design. For instance, a unique doll-shaped 3D structure was self-folded by combining rectangles with cross-shaped hinges, where the rectangles correspond to the body and limbs and a circle forms the head (Fig. 3(b)). The cross portion connecting the arms retained the backbone shape, making it possible to maintain the 3D doll geometry. Hence, by incorporating the branched structure in the pattern interior, it is possible to produce more complex 3D shapes such as dolls, zigzags, and caged skeletal frames.
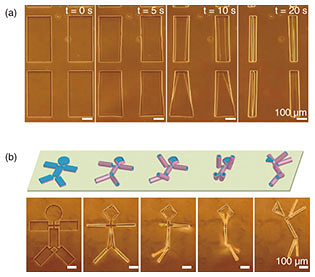
Fig. 3. Using 2D thin films to fabricate arbitrary 3D structures. (a) Fabrication of a cylindrical structure using a rectangular thin film. (b) 3D self-folding with the 3D doll geometry. Reprinted from Teshima et al. [9]. Copyright 2017.
We used Fourier transform infrared spectroscopy (FTIR) to characterize the molecular conformation of the self-folded micro-rolls [9]. Silk fibroin was methanol-treated to induce β-sheet conformation in its crystalline layer. The spectra ranging from 1450 to 1700 cm−1 are assigned to the absorption of the peptide backbones in silk fibroin (Fig. 4(a)). All of the films containing the silk fibroin have spectra centered at 1625 cm–1, which is characteristic of the antiparallel β-sheet conformation in crystalline silk. However, the folded micro-rolls exhibit a reduction in absorbance around 1650 cm−1, suggesting a transition from the helical conformation of amorphous silk to a β-sheet-rich conformation. There are no particular differences in the peak wavelengths of the micro-rolls with varying curvature radii. In addition, thanks to its high elastic modulus, the β-sheet-rich matrix was maintained without a phase transition after the self-folding step. Accordingly, the inducement of the β-sheet-rich conformation is an essential process to maintain the 3D shape of the micro-rolls and make them sufficiently durable for long-term incubation.
We applied quantitative predictions of 2D designs to the final 3D geometry to allow us to control the micro-roll architecture [9]. Since the underlying mechanism of self-folding is the stiffness mismatch in the bilayer, the curvature of the micro-rolls (1/ρ) was calculated using Timoshenko’s bimorph beam theory [14] (Fig. 4(b)). This theory predicts the curvature radius (ρ) based on the elastic modulus, layer thickness, and strain. For simplicity, we assumed a constant thickness of silk fibroin. The experimental value ρ is plotted in the equilibrium state as a function of thickness of parylene from 30 to 400 nm. We confirmed that ρ mostly follows the trend predicted by the bimorph beam theory.
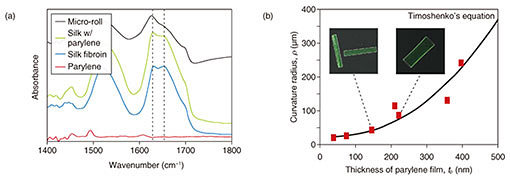
Fig. 4. Characterization of self-folded micro-rolls. (a) FTIR spectra of crystalline silk fibroin in micro-rolls. Resonance absorbances centered at 1650 and 1625 cm−1 respectively indicate a random coil conformation in amorphous silk protein and the helical β-sheet conformation of annealed protein. (b) Plot of average curvature radius of free-floating, self-folded 200×400 μm2 films for various parylene film thicknesses. Reprinted from Teshima et al. [9]. Copyright 2017.
3.2 Encapsulation and manipulation of cells
The key challenge with cell-laden micro-rolls is to fabricate tissue-mimicking tubular structures with non-cytotoxicity and to confirm their cellular function. We encapsulated human embryonic kidney (HEK) cells within the micro-rolls. When EDTA was added, the cells on the 2D film surface were encapsulated inside the 3D micro-rolls through the self-folding of the bilayer (Fig. 5). Confocal cross-sectional images revealed that the arrayed micro-rolls encapsulated numerous cells at a time, which were separated from the cells outside the patterned area. A live/dead assay revealed that more than 95% of the cells were still alive inside the micro-rolls after exposure to EDTA. This indicates that the self-folding and encapsulation process was sufficiently gentle to avoid cell damage. Since the curvature radius and length of the micro-rolls are controllable, the number of encapsulated cells can be easily controlled with the cell density and the volume of the micro-roll. The use of an array of micro-rolls potentially makes it possible to create highly parallel assays that can be used to investigate cellular behaviors including migration, proliferation, and differentiation.
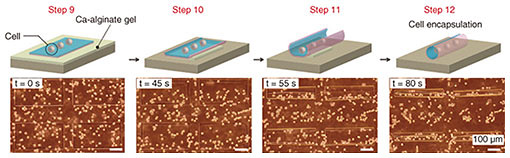
Fig. 5. Encapsulation of cells. As the Ca-alginate gel layer is removed by EDTA, the thin film deforms into a tubular structure. At the same time, cells near the surface of the film are encapsulated inside it. Reprinted from Teshima et al. [9]. Copyright 2017.
The high biocompatibility and transparency of the micro-rolls enable stable culturing of encapsulated cells. During the incubation, the encapsulated cells migrated and proliferated only inside the micro-rolls without any migration out of the micro-rolls. This leads to the formation of cell-cell contacts along the inner surfaces of the micro-rolls. The micro-rolls with encapsulated cells not only provide the transparency needed for microscope observations, but they also have sufficient mechanical strength to withstand the cell traction force. HEK cells exhibit relatively strong cell-to-cell contact, so the cells in a micro-roll aggregated and filled the micro-roll cavity after one day of culturing. Specifically, the cells proliferated, filled the micro-roll cavity, and then migrated onto the outer silk fibroin surface of the micro-rolls. The shapes of the cell clusters differed depending on the micro-roll volume and the cell-line’s adhesive properties.
A pico-liter fluid flow done with a glass capillary enables manipulation of cell-laden micro-rolls [9]. The smooth withdrawal and release of cells from the capillary enables us to relocate cells to the target location (Fig. 6(a)). When the micro-cells were being conveyed inside the glass capillary, the micro-rolls stably maintained their cylindrical shapes without buckling or bending. Therefore, individual cells inside the micro-rolls were collected and transported to the targeted position to induce cell-to-cell contact (Fig. 6(b)). Note that the cells on micro-rolls were manipulated without stripping them from their growth surfaces, which allowed the adhesive state of the manipulated cells to be preserved. This has the potential to facilitate high-order cellular assembly for potential use in tissue engineering by enabling the manipulation of individual cell types into any desired multi-cell-line combination.
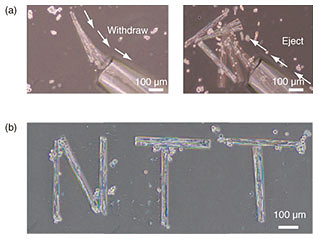
Fig. 6. (a) Manipulation of 400-μm-long cell-laden micro-rolls in culture medium. The micro-rolls can be withdrawn, relocated, and ejected with a picoliter flow using glass capillaries. (b) Micrograph of manipulated multiple cell-laden micro-rolls in culture medium.
3.3 Reconstruction of functional tissue-like structures
Physiological functions within the cell-laden micro-rolls were investigated using two types of primary cultured cells: cardiomyocytes and hippocampal cells [9]. In contrast to the cell lines, neither of these proliferate spontaneously, and their cell bodies tend to reside in the micro-rolls. After five days of culture, the cardiomyocytes aggregated to form a more than 2-cm-long tubular structure. A time-dependent displacement at corresponding pixel locations was estimated by subtracting the average intensity from the optical images (Fig. 7). The intensity corresponding to the displacement shows that all the connecting cells synchronously contracted in the same period at approximately 1 Hz. Time-lapse fluorescence image measurement of fluo-4-labeled cells shows that the oscillation of intracellular Ca2+ propagated and was synchronized within the micro-rolls. The microenvironment provided by micro-rolls of any length was able to regulate the cell migration and cell-cell interactions and drive intrinsic morphologies and functions.
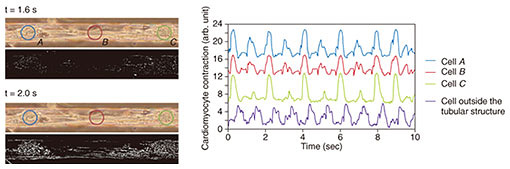
Fig. 7. Reconstruction of minute myocardial tissue structures. (Left) Phase contrast micrographs of cardiomyocytes cultured inside tubular structures, and black and white images showing the displacement of the cells during a heartbeat. (Right) Graph showing the amount of displacement during a heartbeat. In the tubular structure containing the cardiomyocytes, the cells are bound to each other to form a fibrous biological tissue-like structure. The cardiomyocytes beat in unison with a rhythm that is synchronized and constant. Reprinted from Teshima et al. [9]. Copyright 2017.
Primary hippocampal cells were also encapsulated to reconstitute neural tissues. The encapsulated cells aggregated and subsequently extended their dendrites in the micro-roll direction. After three days of culturing, the neurite-mediated cell-cell connection between the paired cell aggregation resulted in the formation of unidirectional neural cell fibers (Fig. 8(a)). Somas and their neurites were both located inside the micro-rolls without any protrusions. Interestingly, the neurites were further extended outside the micro-rolls, where they connected with neighboring cells. Immunostaining of neurites and somas showed that both were positionally restricted within the micro-rolls. Some spheroids were located at the edge of the micro-rolls, and the others were evenly distributed inside the micro-rolls. Regardless of their location, the dendrites were extended and evenly distributed in the micro-rolls after long-term incubation.
To further investigate the accessibility of neurites to the outer surface, we examined cellular responses to external stimulation with added glutamic acid. When the glutamic acid receptors in the hippocampal cells were activated, the intracellular Ca2+ were detectable by calcium indicators using confocal laser scanning microscopy (Fig. 8(b)). Fluo-4 labeled cells exhibited spontaneous Ca2+ oscillations that were synchronized across the cells via synaptic connections (cells H, I, J), whereas the cells that were not connected via neurites showed no oscillation signals (cell K). This result indicates that the encapsulated cells come into direct contact and build up a cellular network with the cells outside of the micro-rolls through hollow structures in order to transmit and exchange information with their neighboring cells. After two weeks of culturing, the extended neurites from the manipulated cell-laden micro-rolls were connected and bridged to achieve cell-to-cell contact between neighboring micro-rolls. Daily medium exchanges and cell maintenance ensures the long-term culturing of encapsulated cells without cytotoxicity. Consequently, the relocated cells can recreate intercellular communication with the cells outside the micro-rolls, serving as building units of higher-order tissue assemblies and potential implantable grafts in vivo. 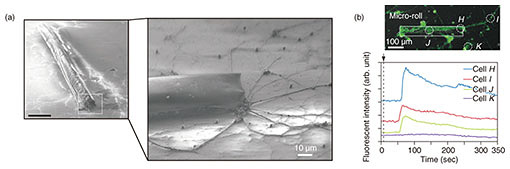
Fig. 8. (a) SEM images of hippocampal cell-laden micro-rolls cultured on poly-D-lysine/laminin-coated dishes. (b) Relative fluorescence intensity profiles of Ca2+ oscillations in hippocampal cells labeled with Fluo-4 and stimulated by adding glutamic acid in the segment shown in the confocal fluorescent image. Reprinted from Teshima et al. [9]. Copyright 2017.
4. Summary
In summary, we proposed that a polymeric multi-layered film transforms into a programmed 3D configuration that depends on 2D geometry [9]. Conventionally, it is difficult to induce a stress gradient in homogeneous films due to weak adhesion in layered hydrogel film [15]. The micro-rolls proposed in this study are improved by coating the film with parylene using chemical vapor deposition [10, 16], which results in the fabrication of single-cell-sized 3D structures with highly precise geometry in the same manner as bimetal films. The self-folding mechanism is based on the bending of bilayer films; thus, it is potentially applicable to any constituent materials.
The process of fabricating micro-rolls does not require cytotoxic etchant, unlike conventional self-foldable inorganic bimetal thin films. This is attributable to the non-cytotoxic dissolution of the sacrificial Ca-alginate layer, allowing for the batch encapsulation of multiple cells. The biocompatibility of silk fibroin and parylene also ensured the encapsulation, long-term culturing of more than one month, and facile manipulation of encapsulated cells without cytotoxicity. Notably, the higher elasticity of parylene and silk fibroin enables the embedded cell to develop a fiber-shaped construct. As a result, the contraction of the primary myocytes and synaptic connections of highly oriented primary neural cells was achieved. The transparency of the polymers used facilitates the study of any cellular response such as calcium oscillation and the fluorescent observation of an immunostained cytoskeleton. This method could be expanded to many other adherent cell lines for the reconstruction of fiber-shaped functional tissues that mimic muscle fibers, blood vessels, and nerve networks in vitro.
5. Future prospects
The system demonstrated here can be used to form scaffolding structures toward regenerative medicine and the behavior analysis of single cells. It also enables encapsulated cells to be freely manipulated in the format of 3D shapes. Importantly, the adherent cells inside the micro-rolls are stably encapsulated and manipulated while retaining their adhesive properties. Although the cell-laden micro-rolls were entirely manually manipulated in this study, our manipulation platform is incorporated with an encapsulated magnet-responsive layer [11, 17] or a microfluidic system [18] for fast, reliable, and automated manipulation. Additionally, the distance-mediated interconnection of elongated neurites between more than two neuron-laden micro-rolls is worthy of a detailed investigation involving optimizing their sizes, location, and patterns from the perspective of building synthetic neural circuits and tissue engineering. This method could be expanded in the future to reconstruct tissues with various shapes in vitro that are suitable for exploring single-cell assays, tissue engineering, and implantable ex vivo tissue grafts.
References
[1] | W. Xi, C. K. Schmidt, S. Sanchez, D. H. Gracias, R. E. Carazo-Salas, S. P. Jackson, and O. G. Schmidt, “Rolled-up Functionalized Nanomembranes as Three-dimensional Cavities for Single Cell Studies,” Nano Lett., Vol. 14, No. 8, pp. 4197–4204, 2014. |
[2] | H. Onoe, T. Okitsu, A. Itou, M. Kato-Negishi, R. Gojo, D. Kiriya, K. Sato, S. Miura, S. Iwanaga, K. Kuribayashi-Shigetomi, Y. T. Matsunaga, Y. Shimoyama, and S. Takeuchi, “Metre-long Cell-laden Microfibres Exhibit Tissue Morphologies and Functions,” Nat. Mater., Vol. 12, No. 6, pp. 584–590, 2013. |
[3] | T. M. Chang, “Therapeutic Applications of Polymeric Artificial Cells,” Nat. Rev. Drug Discov., Vol. 4, No. 3, pp. 221–235, 2005. |
[4] | V. Stroganov, M. Al-Hussein, J. U. Sommer, A. Janke, S. Zakharchenko, and L. Ionov, “Reversible Thermosensitive Biodegradable Polymeric Actuators Based on Confined Crystallization,” Nano Lett., Vol. 15, No. 3, pp. 1786–1790, 2015. |
[5] | O. G. Schmidt and K. Eberl, “Nanotechnology: Thin Solid Films Roll up into Nanotubes,” Nature, Vol. 410, No. 6825, p. 168, 2001. |
[6] | K. Malachowski, M. Jamal, Q. R. Jin, B. Polat, C. J. Morris, and D. H. Gracias, “Self-folding Single Cell Grippers,” Nano Lett., Vol. 14, No. 7, pp. 4164–4170, 2014. |
[7] | Z. L. Wu, M. Moshe, J. Greener, H. Therien-Aubin, Z. H. Nie, E. Sharon, and E. Kumacheva, “Three-dimensional Shape Transformations of Hydrogel Sheets Induced by Small-scale Modulation of Internal Stresses,” Nat. Commun., Vol. 4, p. 1586, 2013. |
[8] | O. Guillame-Gentil, O. Semenov, A. S. Roca, T. Groth, R. Zahn, J. Vörös, and M. Zenobi-Wong, “Engineering the Extracellular Environment: Strategies for Building 2D and 3D Cellular Structures,” Adv. Mater., Vol. 22, No. 48, pp. 5443–5462, 2010. |
[9] | T. F. Teshima, H. Nakashima, Y. Ueno, S. Sasaki, C. S. Henderson, and S. Tsukada, “Cell Assembly in Self-foldable Multi-layered Soft Micro-rolls,” Sci. Rep., Vol. 7, p. 17376, 2017. |
[10] | T. Teshima, H. Onoe, K. Kuribayashi-Shigetomi, H. Aonuma, K. Kamiya, H. Ishihara, H. Kanuka, and S. Takeuchi, “Parylene Mobile Microplates Integrated with an Enzymatic Release for Handling of Single Adherent Cells,” Small, Vol. 10, No. 5, pp. 912–921, 2014. |
[11] | T. Teshima, H. Onoe, H. Aonuma, K. Kuribayashi-Shigetomi, K. Kamiya, T. Tonooka, H. Kanuka, and S. Takeuchi, “Magnetically Responsive Microflaps Reveal Cell Membrane Boundaries from Multiple Angles,” Adv. Mater., Vol. 26, No. 18, pp. 2850–2856, 2014. |
[12] | T. Teshima, H. Nakashima, N. Kasai, S. Sasaki, A. Tanaka, S. Tsukada, and K. Sumitomo, “Mobile Silk Fibroin Electrode for Manipulation and Electrical Stimulation of Adherent Cells,” Adv. Funct. Mater., Vol. 26, No. 45, pp. 8185–8193, 2016. |
[13] | D. N. Rockwood, R. C. Preda, T. Yücel, X. Q. Wang, M. L. Lovett, and D. L. Kaplan, “Materials Fabrication from Bombyx mori Silk Fibroin,” Nat. Protoc., Vol. 6, No. 10, pp. 1612–1631, 2011. |
[14] | S. Timoshenko, “Analysis of Bi-metal Thermostats,” J. Opt. Soc. Am., Vol. 11, No. 3, pp. 233–255, 1925. |
[15] | M. Jamal, A. M. Zarafshar, and D. H. Gracias, “Differentially Photo-crosslinked Polymers Enable Self-assembling Microfluidics,” Nat. Commun., Vol. 2, p. 527, 2011. |
[16] | T. Teshima, H. Onoe, S. Tottori, H. Aonuma, T. Mizutani, K. Kamiya, H. Ishihara, H. Kanuka, and S. Takeuchi, “High-resolution Vertical Observation of Intracellular Structure Using Magnetically Responsive Microplates,” Small, Vol. 12, No. 25, pp. 3366–3373, 2016. |
[17] | S. Tottori, L. Zhang, F. M. Qiu, K. K. Krawczyk, A. Franco-Obregón, and B. J. Nelson, “Magnetic Helical Micromachines: Fabrication, Controlled Swimming, and Cargo Transport,” Adv. Mater., Vol. 24, No. 6, pp. 811–816, 2012. |
[18] | W. H. Tan and S. Takeuchi, “A Trap-and-release Integrated Microfluidic System for Dynamic Microarray Applications,” Proc. Natl. Acad. Sci. USA., Vol. 104, No. 4, pp. 1146–1151, 2007. |
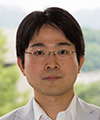 |
- Tetsuhiko Teshima
- Researcher, NTT Basic Research Laboratories.
He received a B.E., M.E., and Ph.D. from the School of Agriculture, Arts and Science, and Information Science and Technology, University of Tokyo, in 2009, 2011, and 2014. During 2011–2014, he was a research fellow of the Japan Society for the Promotion of Science (JSPS). He joined NTT Basic Research Laboratories in 2014 and is currently working on a silk fibroin-based biocompatible interface with skin and in vivo tissue to monitor vital data. He is a member of the Materials Research Society of the USA and the Japan Society of Applied Physics (JSAP).
|
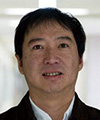 |
- Hiroshi Nakashima
- Senior Research Scientist, Supervisor, NTT Basic Research Laboratories.
He received a B.E., M.E., and Ph.D. in applied chemistry from Waseda University, Tokyo, in 1995, 1997, and 2002. He joined NTT Basic Research Laboratories in 1997. He has since been researching the synthesis and control of optoelectrical properties of (semi)conductive polymers and nano-bio materials. He is a member of the Chemical Society of Japan (and its Colloid and Surface Chemistry Division) and JSAP (and its Molecular Electronics and Bioelectronics Division).
|
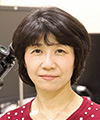 |
- Yuko Ueno
- Senior Research Scientist, Supervisor, Distinguished Researcher, NTT Basic Research Laboratories.
She received a B.S., M.S., and Ph.D. in chemistry from the University of Tokyo in 1995, 1997, and 2002. She joined NTT Integrated Information & Energy Systems Laboratories in 1997. She was a post-doctoral fellow at the University of California at Berkeley and at Lawrence Berkeley National Laboratory, Berkeley, CA, USA, from 2004 to 2005. She is a member of the Japan Society of Analytical Chemistry, the Chemical Society of Japan, the Spectroscopical Society of Japan, JSAP, the Japan Society of Vacuum and Surface Science, the Society for Chemistry and Micro-Nano Systems, and the Institute of Electronics, Information and Communication Engineers.
|
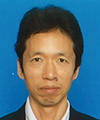 |
- Satoshi Sasaki
- Senior Research Scientist, NTT Basic Research Laboratories.
He received a B.S., M.S., and Ph.D. in applied physics from the University of Tokyo in 1988, 1990, and 1993. He joined NTT Basic Research Laboratories in 1993. Since then, he has been studying transport properties of mesoscopic devices, especially quantum dots. He is a member of the Physical Society of Japan and JSAP.
|
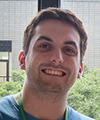 |
- Calum S. Henderson
- Internship Student, NTT Basic Research Laboratories.
He is currently studying toward a master’s degree in the School of Chemistry, the University of Edinburgh, Scotland. He joined NTT Basic Research Laboratories in 2017 through an industrial placement program. He will join the ISIS Neutron and Muon Source, Oxfordshire, England as a researcher in 2018.
|
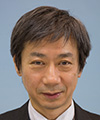 |
- Shingo Tsukada
- Senior Distinguished Researcher, NTT Basic Research Laboratories.
He received an M.D. from Toyama Medical and Pharmaceutical University and his medical license in 1990, and a Ph.D. in medicine from University of Tsukuba, Ibaraki, in 2003. He was a visiting researcher at the University of California at San Diego, CA, USA, during 2003–2005. He joined NTT Basic Research Laboratories in 2010. He has been studying the mechanisms and activity control of signal transduction of brain cells. He is a member of the Society for Neuroscience, the Physiological Society of Japan, JSAP, the Japan Neuroscience Society, the Japanese Circulation Society, and the Japanese Orthopaedic Association.
|
↑ TOP
|